
A strontium magneto-optical trap.
How did a quantum physics experiment end up looking like a night club? Due to a fortunate coincidence of nature, my lab mates and I at Endres Lab get to use three primary colors of laser light – red, blue, and green – to trap strontium atoms. Let’s take a closer look at the physics behind this visually entrancing combination.
The spectrum

The electronic spectrum of strontium near the ground state.
The trick to research is finding a problem that is challenging enough to be interesting, but accessible enough to not be impossible. Strontium embodies this maxim in its electronic spectrum. While at first glance it may seem daunting, it’s not too bad once you get to know each other. Two valence electrons divide the spectrum into a spin-singlet sector and a spin-triplet sector – a designation that roughly defines whether the electron spins point in the opposite or in the same direction. Certain transitions between these sectors are extremely precisely defined, and currently offer the best clock standards in the world. Although navigating this spectrum requires more lasers, it offers opportunities for quantum physics that singly-valent spectra do not. In the end, the experimental complexity is still very much manageable, and produces some great visuals to boot. Here are some of the lasers we use in our lab:
The blue
At the center of the .gif above is a pulsating cloud of strontium atoms, shining brightly blue. This is a magneto-optical trap, produced chiefly by strontium’s blue transition at 461nm.
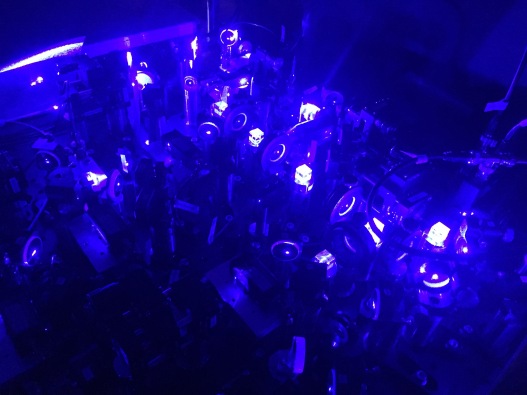
461nm blue laser light being routed through various paths.
The blue transition is exceptionally strong, scattering about 100 million photons per atom per second. It is the transition we use to slow strontium atoms from a hot thermal beam traveling at hundreds of meters per second down to a cold cloud at about 1 milliKelvin. In less than a second, this procedure gives us a couple hundred million atoms to work with. As the experiment repeats, we get to watch this cloud pulse in and out of existence.
The red(s)

689nm red light. Bonus: Fabry-Perot interference fringes on my camera!
While the blue transition is a strong workhorse, the red transition at 689nm trades off strength for precision. It couples strontium’s spin-singlet ground state to an excited spin-triplet state, a much weaker but more precisely defined transition. While it does not scatter as fast as the blue (only about 23,000 photons per atom per second), it allows us to cool our atoms to much colder temperatures, on the order of 1 microKelvin.
In addition to our red laser at 689nm, we have two other reds at 679nm and 707nm. These are necessary to essentially plug “holes” in the blue transition, which eventually cause an atom to fall into long-lived states other than the ground state. It is generally true that the more complicated an atomic spectrum gets, the more “holes” there are to plug, and this is many times the reason why certain atoms and molecules are harder to trap than others.
The green
After we have established a cold magneto-optical trap, it is time to pick out individual atoms from this cloud and load them into very tightly focused optical traps that we call tweezers. Here, our green laser comes into play. This laser’s wavelength is far away from any particular transition, as we do not want it to scatter any photons at all. However, its large intensity creates a conservative trapping potential for the atom, allowing us to hold onto it and even move it around. Furthermore, its wavelength is what we call “magic”, which means it is chosen such that the ground and excited state experience the same trapping potential.

The quite powerful green laser. So powerful that you can see the beam in the air, like in the movies.
The invisible
Yet to be implemented are two more lasers slightly off the visible spectrum at both the ultraviolet and infrared sides. Our ultraviolet laser will be crucial to elevating our experiment from single-body to many-body quantum physics, as it will allow us to drive our atoms to very highly excited Rydberg states which interact with long range. Our infrared laser will allow us to trap atoms in the extremely precise clock state under “magic” conditions.
&
The combination of strontium’s various optical pathways allows for a lot of new tricks beyond just cooling and trapping. Having Rydberg states alongside narrow-line transitions, for example, has yet unexplored potential for quantum simulation. It is a playground that is very exciting without being utterly overwhelming. Stay tuned as we continue our exploration – maybe we’ll have a yellow laser next time too.
&
Comments